Flash and JavaScript are required for this feature.
Download the video from iTunes U or the Internet Archive.
Topics covered: Diploid Genetics
Instructor: Prof. Graham Walker
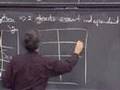
23: Diploid Genetics
I just got the feedback from the one minute things just a few minutes before class, so I think I may defer commenting on the couple of them until the beginning of next lecture. But there are a couple of things that I think I can say. One is, several people were wondering, how does the cell decide whether to do mitosis or meiosis?
But the mitosis, which is ordinary cell division is what happens everywhere in your body, in your intestine, on your skin, in your eye, anywhere except, since that I you make all the different cells you have. The meiosis, which creates the sex sells, or gametes, happens in a very specific place, either in the testes if you are male or the ovary if you are a female.
So, there's a dedicated place where meiosis takes place, and just your knowledge of human anatomy and physiology can make a pretty good guess as to where that is. Everywhere else, it's mitosis.
There's a very special place where that happens. A couple of you still got confused when I was talking about meiosis and I were showing you a progression through, and I put a double headed arrow, meaning that's was with that period was called. The process is unidirectional. It only goes in one way.
It's not a reversible process. There were some questions about the chiasmata or the chiasma: why is there a crossover in chiasma in meiosis but not mitosis? I will tell you about that today very specifically. Why was there a tall and a short pair of chromosomes? That was arbitrary. I made up a simple cell for us to consider these properties in, one with a long, and what with a short, nothing else. I wasn't trying to represent any particular organism.
And I'll pick up on a couple of other things. I just want to quickly mention something. Since I've seen you, I flew out to San Diego, gave a talk yesterday morning at a major meeting, hopped on a plane, got back at midnight, and here and again.
As part of being a scientist, come teacher, at a place like MIT, you guys tend not to see, but my research life goes on while I'm teaching. And I just want to briefly mentioned one thing I talked about yesterday, because just in my own life it captures a couple of things that I've been trying to tell you, that the textbook is not the ultimate authority.
That's just what we think up until today. A new finding to change the way you think about things, and what I'm telling you, and some of your frustrated at, that I'm not just parroting back the textbook is because this is the way it is. If you guys are going to be leaders in whatever field you're in, you're going to be dealing with this process of shifting sands as we gain new knowledge.
So, in fact what I was talking about has some relationship to the cell cycle that I talked about. Lee Hartwell particularly helped us understand that there is what's called a G1 phase, which you could think of as a preparation for DNA synthesis for what's known as the S-phase where DNA synthesis actually occurs.
And now, you're at 4N. You've doubled the DNA content, and there's G2 where it cleans up from S-phase, gets ready for mitosis, and mitosis is what you, then, separate the daughter chromatids, go back to 2N, and then ultimately the cell divides.
And we are back to 2N. And what we were talking about DNA replication, I told you how replicative DNA polymerases test for Watson-Crick shape, and remember that little movie I showed you where they flipped the base pair into a very narrow slot in the protein, and they check that it's there. And they said at that time, that's why polymerases have a problem when they hit a lesion such as a thymine dimer that we get we go out into the sun.
And then one of the recent pieces of excitement in the DNA repair field that I work in, was the discovery of a whole class of translesion DNA polymerases that are very flexible, active sites and we are able to copy over a lesion.
Right at this point, you will find in the literature all sorts of reviews about polymerase switching, where people are envisioning the replicate of polymerase coming along.
It hits a lesion, gets stuck. It recruits one of these translesion polymerases that comes in, copies of the lesion; it switches back to the replica of polymerase, [and then it goes?]. And there are reviews like that coming out in the literature. So, one of the genes that's needed for this sort of error prone kind of translesion synthesis in yeast and in humans is a gene called [rev-1?
. You don't have to notice for the exam, this bit.
But if you knock out the function of that gene, the yeast aren't mutated by UV or chemicals anymore. So, you know it has an essential function somehow in this process of translesion synthesis.
So, one of our big surprises was I was trying it.
We were trying to actually fish out partners that might interact with it cause we thought it would be regulated. And we were surprised.
The experiments revealed something we hadn't expected.
We found out that this protein that was critical for mutagenesis was extremely strongly cell cycle regulated.
Well, given the reviews I've told you, or if somebody you're writing a textbook today, this is what they would tell you: polymerase switching during S-phase. So you might have thought it would be high and S-phase, but it isn't. We can barely detect it during S-phase. But instead, the rev-1 levels are at least 50 times in the G2M phase. Actually, it goes right through this part of the cell cycle, 50 times what they are during S-phase. And so, there's a couple of possibilities right now. Either these very tiny amounts that are doing S-phase are what everybody thinks and current models would predict: you're getting polymerase switching. And it makes 50 fold more during this phase for something else. If so, I don't know what that is. The other possibility is that we have to rethink our model, and that it isn't polymerase switching during replication.
Actually, the replication for it just keeps moving, and it leaves behind little masses. And when the cell is busy starting to line up as chromosomes, and even while it's pulling them apart, that's when this translesion synthesis stuff comes in, and it cleans up the damage. Now, I don't know what the right answer is. That was one of the things I was talking about yesterday morning. But it's an example of how something that you guys can now hopefully at least understand in principle is being debated right now. And the finding from my lab changed the way I'd been thinking about it at least. I can now see another very real possibility. So, just to move on, so we are going back to Mendel now, who did an awful lot more than I told you. A few of you thought it was pretty frustrating and it had all that. But Mendel was doing other things. You already know what he could do. He knew how to do crosses. He knew how to count.
And he could think, which was really important.
And he's our ratios instead of just numbers. So it was another class of experiment that he could do, and that was he could do a cross where he looked at more than one trait at once.
And he didn't have all that many options for things he could do, but he did what are known as dihybrid crosses, where he followed two traits at once. And I showed you some of the traits that he studied. In fact, that picture I showed you is actually a cross that we can think of right now.
He's got smooth, and yellow, wrinkled, and green, and yellow, and you'll see them in all four combinations. So, an example of the kind of crust that Mendel carried out, then, was he took smooth yellow, which actually is the dominant allele, and [both? , which he learned from his other crosses. And he crossed it with wrinkled green, which I'll represent as a little s, little s, little y, little y, where he previously known the little s and little y alleles, the wrinkled and green were the recessive alleles. And then, the F1 generation, wouldn't be surprising to you at this point, I think, that they were all smooth, yellow. And, they all were ss yy.
And if you think about the possible gametes you get out of this, you could only get a big S, big Y out of this one, and little s, little y out of that one.
So if they came together, it would have to be that. So then, what he did the self crossed the F1, and what he got out of that was the kind of mixture of things that he saw there.
He saw smooth yellow, smooth green, wrinkled yellow, and wrinkled green. In doing the same kind of experiments that we talked about before, he counted them, he looked to see if he saw characteristic ratios. He did.
The ratios he saw were 9:3:3:1. So, I think we know how his head worked at this point. He was try to figure out if he could explain these results by the kind of model that he was developing where hereditary information came in, in particles. And he could.
But he had to make a critical assumption.
And if you've just seen the next square going to draw, without realizing the assumption underlies it, then you've missed a great deal of his thinking. And that was that the two genes of sort, or the two traits anyway, because he didn't know they were genes yet independently. And the way we could see that would be to think about what were the kind of gametes that you could make from those F1s. So, we could get a big S and a big Y from here, or a big S and a little y, or a little s and a big Y, or a little s and a little y. And, since this is [selfing?
, it's the same thing. So I won't fill this whole thing in, but we get two S's, two big Y's here. Down here we'd have little s, little y like that. I'll fill in a couple up here where we've got big S, big S, big Y, and a little Y. Here we have big S, big S, but two little Y's. Here we'd have big S, little s, big Y little y. And over here we'd have big S, little S, two little y's. So, if you were to make out [then?
a table or a chart like this that showed the phenotypes, you'll find that all of these wild type, we can see it here.
However, this one would be smooth. But it's got the two alleles. So it would be green. This one would be smooth and yellow.
And this, again, would be smooth but green.
And down on the corner it is the wrinkled green.
And if you follow that out, draw out the rest of that, you'll discover there the 9:3:3:1 ratios. That was what Mendel observed in all of his experiments. But he was particularly bothered by it because he'd shown particular information. The fact [they sorted?
randomly wasn't an issue. However, that was before people knew about the chromosomes, which we spent quite a bit of time talking about the other day. As I said, when people saw those chromosomes that gave rise to what's known as the chromosomal theory of inheritance. And what was interesting about chromosomal theory of inheritance was that it predicted a different outcome depending on whether the traits you were studying were encoded by genes on the same chromosome or by genes on different chromosomes. Now, as it happened, what I think Mendel did was he found traits that were well behaved and he could study.
And those all happened to be on separate chromosomes.
So, his results didn't disclose this issue that was raised by the discovery of chromosomes. The chromosomal theory of inheritance gives, as I said, predicts different outcomes depending whether things are on the same or different chromosomes. So let's consider that by taking the F1. So, say they are on separate chromosomes. And let's take the F1 from the previous cross over here. So, big S., little s, big Y, little y, and then we'll cross it with the homozygous recessive parent.
That was a cross that I mentioned the first lecture was very important.
It's important enough that it's given a special name.
It's called a test across. Well, here we are using it here.
Well what are the possible outcomes that could come from this sort of thing? Well, I think the way you could think about it most easily is, what kind of gametes or sex cells could we get out of this?
Well, from here we could get a big S and a big Y. Or, we could get a big S. and the little y, a little S and a big Y, or a little S and a little y. So, we could get four different types of sex cells. The gametes we can get out of this sign, there's only one type. So, if we start combining those, I think you can see what the outcome would be.
It's so simple. We don't even need to draw out the square. If we had big S, big Y over, they're all going to be over. Well actually, I'll draw up this way because I think it's a little easier to see. So, there would be four possible things that would come out of this test cross.
This would be the smooth and yellow. Here would be, what have I done wrong here? No, this is right. This should be smooth and green. This would be wrinkled and yellow.
And this would be wrinkled and green. And, do you see what the ratio would be? It would be 1:1:1:1 because we have an equal probability of making any of those.
And, I'm going to rearrange these because the way that it'll help us think about it, this is one of the types that we've found in the original cross. That's a parental phenotype, smooth and yellow. It was one of the parents up there.
This is the other one that looks like one of the original parents.
So, I can divide these into parental or nonparental phenotypes.
These are 1:1, and then these others were ones where the progeny differed from the parent. And they're all 1:1:1:1.
Now, that's what the chromosomal theory of inheritance would predict if they were on separate chromosomes. That's what Mendel saw when he was doing his crosses. What if they were on the same chromosome? So, we are going to have to go back to the original cross now to think this one through. So, what Mendel started with was a smooth, yellow parent.
So that was SYSY. But because these are on the same chromosome, I'm going to depict them this way so we can see they're going to travel as a unit. So that was then crossed with the wrinkled green, which would be a little s, little y. In each case, [UNINTELLIGIBLE] should be F1 in this case will be all smooth and yellow.
The recessive traits disappear, but this time they're only, what the progeny will have, they will have gotten one of the possible alleles from this one, which would be the SY on the same chromosome, and one from the other parent, the little s, little y again on the same chromosome and one from the other parent, the little s and y, again, on the same chromosome.
So now if you start to ask, what will happen if I do a test cross, you're going to get a different outcome. So here's the test cross in this case. So, we've got this F1.
It's smooth and yellow, but it's actually at the chromosomal level now. It's like this, and we're crossing it with the homozygous recessive parent that's now going to look like that.
So if you think through, what kind of gametes could we get out of this?
Well, there's only two possibilities.
We could get this one or this one.
And over here, the kind of gametes we could get, there's only one type. So, what are we going to get out of this cross?
What we'll end up getting is big SY, over the little sy, or little sy over the little sy like that. That's smooth and yellow, wrinkled and green, the ratio of these 1:1.
But you see the difference from what we saw before?
These are the parental phenotypes.
The chromosomal theory of inheritance doesn't predict that you will get, or it predicts that you will not get the nonparental phenotypes. So, if you wanted to distinguish between these two hypotheses, and figure out where your genes were, you'd do the experiment.
And what happened when this was done, again, what often happens in science, you think you've got it straightened out.
You got hypothesis A and hypothesis B, and you do experiment planning to do the scientific method and show it. And you get a result that isn't what you expected with either model. And that's in fact what happened in this case. And it led to the discovery of genetic recombination. And to show you actually the experiments where that was discovered, I'm going to switch to another widely used genetic model, which is the Drosophila melanogaster, or the fruit fly, which you see in the summer around rotting fruit. Or if you're in the biology building, one is apt to land on your sandwich because there's just a few stragglers that get out of the Drosophila genetics labs.
But the one thing you can see from this is the eyes, pretty cool. It's got red. The wild type is a red eye, and the body's brown. And, you can see the red eyes.
If you look at a fruit fly carefully, you'll be able to see, the summer if you take a look, you'll see that they have red eyes.
So, they've been a very useful model organism for genetics partly because they grow pretty fast and they are easy to handle in a lab.
And so, I'll introduce you to a couple of features.
So, the wild type you find in nature is a brown body.
We'll call it a plus, and it has normal wings, and which I'll refer to as plus. And some mutant phenotypes that geneticists have been able to find is a black body which I'll refer to as little b.
And vestigial wings, which I'll refer to as VG.
So, these are fairly easy to score. They look like little tiny wings.
It's fairly easy if you're crossing Drosophila, and that looking at progeny to go through and score, what color are their eyes? And do they have ordinary wings or little wings?
So, this is the kind of cross that was carried out.
We are going to switch now to an organism that has male and female.
Up until now, plants are both. They make pollen, and they have the eggs that are going to develop into the seeds once they're fertilized. But, Drosophila are more like us.
They come in males and females. So, we're going to have to specify which is which. And I think most of you are probably familiar with this terminology. This is the symbol geneticists use for female. And this is the one that is used for male.
So, in this case, let's set up exactly the same kind of thing that we start here. We're going to cross a homozygous parent versus a homozygous recessive parent. That's just exactly the kind of thing we have up here. But I'm going to do at this time using Drosophila genetics speak. And so, we'll be taking female whose wild type for both traits, we're going to cross with a male that's homozygous recessive for both traits. This is the way Drosophila geneticists tend to represent this kind of thing.
So it's exactly the same kind of cross we got before.
And at this point, what we get out of the F1, it shouldn't be news for you. You can see genetically what it's going to be. They'll have to get one allele from here, one allele from there. So, by definition, the only possibility that you can get out of this, the F1s, will all have one allele dominant, and one recessive allele just like up there. And this will be, since the wild type is the dominant allele, this will be brown and normal, and normal wings.
And then, at this point they then will set up a test cross, exactly the same idea. We're going to take the F1.
And in this case, we'll take a female whose got this, and we'll cross her with a male who is homozygous recessive.
So that will be black over black, and vestigial over vestigial. And out of this, there are four possibilities.
We can get parental phenotypes. So, there will be, from that, black over, if you look to the various combinations of how these things could go together, you'll find we can get vestigial plus. Or, we can get black over black, and vestigial over vestigial. For example, this one, coming from those two getting together, and this one coming from this one and this one getting together. So this is just exactly the same kind of thing we've done but done using Drosophila traits.
And then, nonparental, so now we get, for example, black over black, and vestigial over plus, black but normal wings, or we could have black over plus, vestigial over vestigial, which would be a brown body, but with vestigial wings.
So, if we were trying to do this kind of cross, we are doing this kind of cross, and we are trying to see what the outcome would be, Mendel, every trait [assorts?
independently would predict the 1:1:1:1. And the chromosomal theory of inheritance would predict we'd get 1:1 here, and we wouldn't see those at all. Instead, what happened when this experiment was done where numbers that didn't really fit.
This part works pretty well. These are numbers from an experiment of this type. So these are pretty close to 1:1.
That's OK. That fits with both of the models. The surprise is over here where there is [2/6 to 185? . So, these are in the ballpark of 1:1. But what you can see is the result is not what was predicted by the model. This is the kind of thing I've sort of been trying to tell you through the course. It keeps happening in biology. It's not a QED sort of thing where you can work it out by logic. Very often, you do an experiment, you get a result that doesn't fit within your current framework.
And then you have to go back and redo your thinking.
So what was going on here, as it turned out, and it all got sorted out, was scientists had just discovered genetic recombination.
And here is where, what was going on. So when we talked about Meiosis I, and we had duplicated the DNA, so we now had to chromatids, and I showed you the chiasma when it was drying, it looked sort of like this.
And, I said that you always sought these physical attachments that once the homologous pairs were duplicated, they would always be in contact.
And I said there was actually a physical interaction going on there.
Well what was going on in there was an exchange, a recombination event at the DNA level that's just exactly equivalent to what was happening when I showed you the phage cross.
Remember, we had a phage cross, and if we got a recombination in between the two genes, then we could get progeny that were a mixture of the two traits. So, if we were plus plus, for example, these are on the same chromosome. And the other one is like this, black, vestigial, black, and vestigial.
If we get a genetic recombination going on here where these two intersect and recombine in between these two genes, what you get out of that, then, is this chromatid is still the same. It's got both wild type alleles. But in this case, now, the black allele has moved from here to the tip of this one, pairing it with that. And the other chromatid over here, the other homologous pair, now has the plus together with the vestigial here and then here. So, if you, then, draw out the possible gametes one will look like one parent. One will look like the other parent. This one can give rise to a nonparental phenotype in a test cross. And that one can give rise to a nonparental phenotype and a test cross. And that's what's going on in this experiment I've described. But the nice thing about this since this sort of thing is happening is one can calculate, then, a recombination frequency in just the same way that we calculated it when we were doing phage cross. And in this case, it was the recombinants, which in this context I'm referring to the nonparentals, the recombinants over the total of the parental types and the nonparental.
So in this case, this would be 206 plus 185 over 965 plus 944 plus 206 plus 185. And if I haven't blown the arithmetic, that would be a recombination frequency of 17%.
Now, we were back talking about that phage cross.
Remember, we did a pair of crosses between gene one and two, and between two and three, and then we were trying to figure out if they were in the linear order, how we could explain it.
And we finally worked out the order by crossing allele one and three.
And we made a little genetic map that could show the order of gene 1, 2, and 3, based on nothing more than the recombination frequency.
So, that's exactly what people were able to do by this kind of measurement. And that, then, led to the generation of chromosome maps. This is a publication from Science in 1994. This is before the human genome was done. And they were mapping a kind of genetic marker that we'll talk about [when?] we do restriction enzymes. But they were able to associate it with [banning? patterns they had seen on the chromosome. This is the sort of thing that cytologists saw, and as scientists were working this out, then they were able to associate these genetic maps of loci, and begin to associate them with the physical maps of banning patterns [in?] chromosomes. Now we have the sort of ultimate genetic map, which is the sequence of the human chromosomes.
So now we know exactly, to the base pair, how far different genes are.
Part of the way that the human genome was assembled from all these little tiny fragments of DNA that were sequenced were taking advantage of these kind of maps that told the scientists assembling all these little fragments of DNA sequence what order they had to be in, what part of the chromosome they were on, and that kind of thing.
There is, this, then, leads us, though, to other issues since I've now started to talk about chromosomes. And one more thing, let me just say before I leave this. So, from this kind of thing, if the recombination frequency is much less than 50%, then they're on the same chromosome. And the word geneticists use to describe this is they say genes are linked. If the recombination frequency is 50%, then they are on different chromosomes. At this point, it's just random assortment. [You go?] one way or the other [as you get?] a number of 50% if you do this kind of calculation.
And these are referred to as unlinked. And those of you who are thinking about it can probably imagine that there might be a problem, that if you had a very long chromosome, so the two genes you are studying were very far apart, you might get so many recombination events in between that it would begin to look, the recombination frequency might come close to 50%.
And you would perhaps have a difficult time in that genetic cross telling whether the genes are really unlinked. They were on separate chromosomes, or they were linked but very far apart.
So this kind of thing could be a little hard to resolve that kind of situation. But there are other things you can do to look at that.
So, the chromosomes we've been talking about are what are known as autosomes. These are identical pairs.
But there's an exception. And those are the genes that are involved in sex determination. It's the chromosomes that are involved in sex determination. These are known as heterozomes.
And, they're on this picture that I showed you where they used this technique of chromosome painting to show it.
You can see how all these autosomes are in identical pairs.
But this is a male, obviously, because there's the Y-chromosome, and there is an X. If you're a female, you'd have two copies of the X, something I think most of you know. So, if we think about how this works in humans, females have two X's, and males have an X and a Y. In Drosophila, the fruit fly, it's the same thing. Males have two X. Females have two X's, and males have an XY. But there isn't anything magic in nature about females having two of the same, and males having one of each because in birds where it's different enough they use different notation. The females have one of each.
And let me make sure I got my notation right.
I think it's ZZ, excuse me. And the females have one of each. So, nature tends to use these differences as far as sex determination. And this, then, poses a new kind of problem. And that is, what would happen if you were doing a cross, and the allele that you are studying happened to be a sex chromosome instead of one of these?
You might guess that since females have two of one and males have one of each that I would not give the results that are predicted by what we've talked about so far. And so, this led to the discovery of what's known as sex linkage. And that's important.
And in fact, as you'll see in a minute, affects stuff that we are familiar with in our lives. I want to just quickly introduce you to this, and show you how it was discovered. It was done by Thomas Morgan in 1910 actually, this discovery. What he was doing was he took a white eyed male, crossed it with a red eyed female wild type, and yet the expected result that the F1s were all red eye. But then when he took the F1 female, which was red, and crossed it with a red eyed male, you got something that was very puzzling at the time.
The females were all red. The males, half of them had red eyes, and half of them had white eyes. If you followed the logic up until now, where you try to work this out, you would find that you couldn't generate this pattern by the stuff that we've talked about now. So once again, this led to the need to create a new model, something that expanded our thinking.
So, the way the thinking went was, well, there must be something to do with the sex of the fruit fly in this. And so, here was the hypothesis, and that was that the white eyed male had this genotype. They had an allele that caused the white eyeness. But it was located on the X chromosome so that the male would have had a Y chromosome paired with that. And, the red eyed female used in the first cross would have a wild type allele on both X chromosomes.
And so, if that were the model, what's going to happen to them when we do this cross that we've described here?
Well, let's think it through. So, we've got female whose X plus.
We're crossing with the male who's got the X with the white allele over the Y. So, the females can either be, they'll get an X plus XW, and the males, yeah, right.
The males are going to get, they will get this allele for Y.
So now, if he takes us red eyed female, that was the F1 from the cross up here, which will be this, and crossed with a red eyed male. Now, that means the male has to have the good allele. What are we going to get?
Well, for the females in this cross, we've got a couple of possibilities.
This one, we could get just the wild type female back, or we could get this one pairing with this one, which will give us this. So, these are all red that fit.
But, the males, then, if you see what happens, they have to have, each have to have a Y, and then they can either get this allele or that allele. If they get this allele, they're red. If this allele, they're white. If you stand back and look at that, you will see that is the outcome that was observed. Females were all red.
The males are half red, half white.
Well, this is a characteristic of, this would be an X-linked trait.
And I want to just point out one thing. This female, excuse me, wrong female. This female here is what's referred to as a carrier. She's got this allele that causes a white guy, but she's not expressing it herself.
But she's able to transmit it to her sons. And when she has progeny, on average, half of her sons will have the trait.
And now, X-linked traits, there are a number of them that we know about. Some of you may know hemophilia. Queen Victoria was a carrier of this gene causing hemophilia, where there is a problem with the clotting mechanism. And if you get a cut, then you can bleed a lot. So, some of her sons had this.
A more common one, which has to apply to some people in this room, is red-green color blindness. If you're a male, you have a much higher probability of being colorblind because it's an X-linked trait. And I want to just close by showing you how human geneticists think about the sort of thing.
And we have to think about things differently if we are doing human genetics because as most of you know, I think all of us would be very uncooperative subjects in a kind of genetic cross that a fruit fly geneticist [or most? geneticists would like to have us do, in which we'd be put in a cage with a member of the other sex and say, mate. That was your choice in life.
Well, that would be a different kind of existence for us all.
So, human geneticists don't have that luxury of having pure breeding strains and doing controlled crosses.
We all have very strong feelings about the kind of crosses that we want to engage in. And so, what they have to do, they have to make use with what they find. And they use a couple of symbols here that I'll just show you. They look at pedigrees, and then they look for patterns. And, they use a little shorthand for doing this. Males are squares, which I don't know if there's any symbolism to that or not, but if they are affected they show it as a shaded square, and unaffected as an open symbol. So, affected males are solid squares.
Affected females are solid circles. So, let's take a look at the sort of pedigree that human geneticists might see. And let's consider something. Let's consider red-green color blindness, which is an X-linked trait. So, let's take a male, which there is probably one in this room at least, maybe more, who have this.
So, since the colorblindness trait is on the X chromosome since he's a male, the other pair will be Y. That means if you're a male and you've got it, you're going to display the phenotypes. So this is, George, let's say, who is colorblind.
They leave a lot of the romance out of these things, as you'll see, who had progeny with Mary. Let's say, they got married after they graduated from MIT.
It was very happy. They had a, let's see, son. He had to get the Y from dad. So, he had to get a good allele from his mother. But they also had a daughter, and she had to get the color blindness allele on an X chromosome because the dad only had one of them, and then a good one from her mom.
So at this point, everybody's normal.
But you'll notice, this daughter has this trait of being a carrier because even though she doesn't display the trait herself, she's got it in her genome. So, let's say, [UNINTELLIGIBLE PHRASE], a woman who doesn't have any colorblindness allele.
So, if we have, let's say, a daughter, and a son, a daughter, and a son, this is not much happening over on the part of the pedigree. Everybody would be normal.
Yeah, did I miss something? Pardon? Oh, it's over Y. Excuse me, yep, I'm going too fast here. Over Y, here we go. I don't need to introduce any genetic abnormalities on top of what we're already trying to do here. This is complicated enough.
OK, so what happens, let's say then that the daughter that married a guy, and they had four children.
I'm going to help us with the genetics of it, from the geneticist, that sort of a perfect family in this case would be four kids representing all four possibilities.
And so, first off let's think what would happen with the daughters.
Well, the daughters could either have the colorblindness allele paired with this one. Or, they could get a wild type allele with this one. So actually, I'd better put this in.
So this is a daughter. So this would be [CB?] over plus, or it could be plus over plus. This one would, again, be a carrier. Now, with the sons, they're both going to get the Y since they're male. But there's a possibility of either getting the good allele, which means he'll be unaffected.
But, if you get the other one, he'll be affected.
Now, this would be sort of a typical pedigree. And you realize, depending on the number of kids, you might or might not see this.
But this, you're going to get to do some more of these and to do some other traits. But let me just sort of point out, if you're a human geneticist, what you would recognize here, the trait's more frequent in males. The frequency of color blindness is about 8% on the X chromosome. So, if you're a female, you've got to have two of them. So that means, you've got 0.64% because you've got to get two together. It's a much smaller probability. The trait skips a generation, [you'd often say?].
You see it here. You see here. But you don't see it in between, and that's because you have this carrier.
The affected males don't transmit to their sons because what they give to their sons is the Y. So, they [kept giving?] the colorblindness thing. And then, the heterozygous females who are carriers will transmit the trait to their sons about half the time. And that's a pattern that a human geneticist would look for.
And they'd say, ah-ha, it must be an X-linked trait.
And you'll see in your problem sets and [recitation?
sections are the patterns. OK, I'll see you on Friday.